
Seal The Entry, Win The War: Mucosal Vaccines For A Safer Tomorrow
Leonardo Padilla Pérez 1, Nur Ain Mohd Asri 2, Min Xuan Keh 2 , Frank Camacho1*.
1 Combinatorial Biology and Bioprocess Laboratory, Department of Pharmacology, School of Biological Sciences, Universidad de Concepción, Concepción, Chile
2 School of Health Sciences, Health Campus, Universiti Sains Malaysia, Kubang Kerian, 16150 Kota Bharu, Kelantan, Malaysia.
* Correspondence: fcamacho@udec.cl;
ABSTRACT
Keywords: mucosal immunity, secretory IgA, vaccine delivery systems, adjuvants, mucosal vaccination strategies, oral and nasal vaccines.
INTRODUCTION
Mucosal surfaces—including the respiratory, gastrointestinal, and genitourinary tracts—serve as the primary entry points for over 90% of human pathogens 1. While parenteral vaccines have revolutionized disease prevention, they typically fail to induce robust mucosal immunity, particularly the production of secretory immunoglobulin A (SIgA), critical for pathogen neutralization at mucosal barriers 2,3. This gap has fueled growing interest in mucosal vaccination strategies that target these frontline tissues directly.
Mucosal vaccines offer several advantages over conventional intramuscular immunization, including eliciting local and systemic immune responses, reducing pathogen transmission through sterilizing immunity, and enhancing memory B and T cell responses 4. Additionally, their non-invasive routes of administration—oral, nasal, sublingual, or rectal—simplify mass immunization logistics, minimize needle-associated risks, and improve patient compliance, especially in pediatric and needle-phobic populations 5,6.
In addition to their immunological advantages, mucosal vaccines present logistical and practical benefits that enhance their applicability in real-world settings. By avoiding needles, mucosal administration reduces the risks of needlestick injuries and bloodborne pathogen transmission while simultaneously minimizing psychological barriers associated with injections 7,8. This ease of administration makes them ideal for mass vaccination campaigns, particularly in low-resource settings or during pandemic outbreaks where rapid deployment is essential 9.
Immunologically, mucosal vaccines stimulate the production of secretory IgA at the site of pathogen entry, creating a robust first line of defense that blocks infection at the mucosal surface before systemic dissemination occurs 10. These vaccines elicit systemic IgG responses and promote the development of long-lived memory B and T cells, offering comprehensive protection 11. This dual response is particularly important in respiratory and enteric infections, where early neutralization is critical to disease control.
Furthermore, mucosal immunization activates the common mucosal immune system (CMIS), allowing immune cells primed at one mucosal site to home to distant mucosal tissues, thereby enhancing cross-site protection 12. This unique property opens new avenues for non-invasive immunization against sexually transmitted infections, gastrointestinal diseases, and respiratory viruses with pandemic potential. As a result, mucosal vaccines are promising as stand-alone strategies and powerful boosters that enhance systemic and mucosal immunity in heterologous prime-boost regimens.
Despite this promise, mucosal vaccines face significant challenges, such as antigen instability in harsh mucosal environments, enzymatic degradation, and poor uptake by antigen-presenting cells 13. Recent innovations in adjuvant development, delivery platforms (e.g., nanoparticles, liposomes, bacteriophage-based carriers), and mucosal immunology are rapidly reshaping the landscape of mucosal vaccine design 14,15.
Given the rapid progress in this field, there is an urgent need for a consolidated synthesis of recent advances to inform research priorities and clinical translation. In this review, we critically evaluate emerging mucosal vaccine platforms, assess their technological promise, and examine key challenges that must be overcome to enable widespread implementation (Figure 1).
Licensed Mucosal Vaccines
Only a limited number of mucosal vaccines have received licensure for human use, primarily via oral or intranasal routes. These include vaccines targeting enteric pathogens—such as Vibrio cholerae (Dukoral®, Shanchol®), Salmonella typhi (Vivotif®), and rotavirus (Rotarix®, RotaTeq®)—as well as respiratory viruses like Influenza A/B (FluMist®) and SARS-CoV-2 (iNCOVACC®, Convidecia Air®) 16,17. The global introduction of rotavirus vaccines alone has prevented an estimated 200,000 child deaths annually and reduced diarrhea-related hospitalizations by up to 84% in some countries 18. Similarly, intranasal influenza vaccines have demonstrated up to 93% efficacy in children in certain seasons, outperforming inactivated injectable vaccines 19,20.
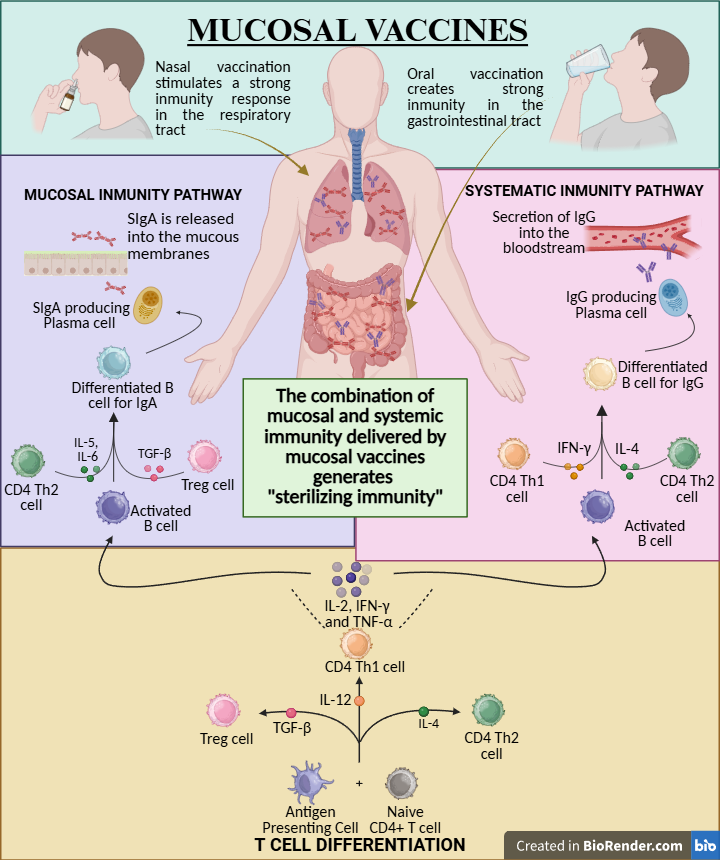
Figure 1. Overview of immune mechanisms triggered by mucosal vaccines. Mucosal vaccination activates both secretory IgA-mediated immunity at the mucosal surface and systemic IgG-mediated immunity, promoting CD4+ T cell differentiation and the development of sterilizing immunity.
In the veterinary field, mucosal immunization has been widely adopted due to its ease of administration and impact on disease control in livestock and wildlife. For example, through bait-based immunization campaigns, oral rabies vaccines (e.g., RABORAL V-RG®) have led to near-elimination of rabies in foxes and raccoons in parts of Europe and North America 21. These successes underscore the real-world potential of mucosal vaccine platforms and provide a valuable foundation for expanding their use in human populations.
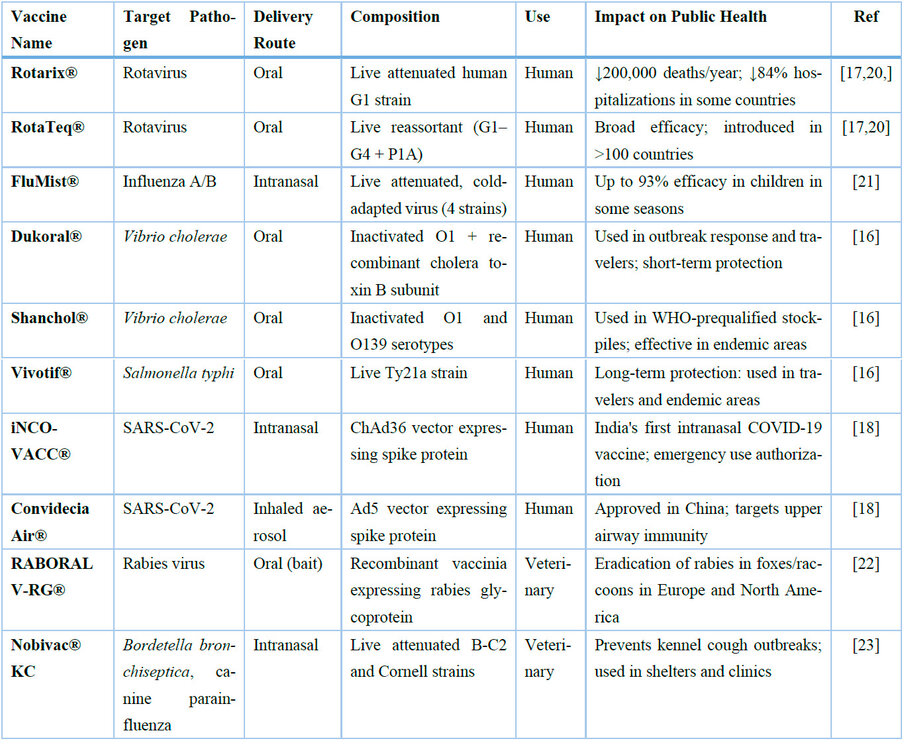
Table 1. Summary of licensed mucosal vaccines approved for human or veterinary use, including delivery routes, antigen composition, and real-world public health impact. This expanded version highlights clinical applications and measurable outcomes in disease prevention programs.
Adjuvants and Delivery Systems for Mucosal Vaccines
One of the primary challenges in mucosal vaccine development is overcoming the biological barriers of mucosal tissues—such as enzymatic degradation, low pH, mucus trapping, and epithelial impermeability—which significantly reduce antigen stability and uptake 22. To enhance immune responses, mucosal vaccines increasingly rely on adjuvants and delivery systems (Figure 2) that protect antigens and promote their interaction with mucosa-associated lymphoid tissues (MALT).
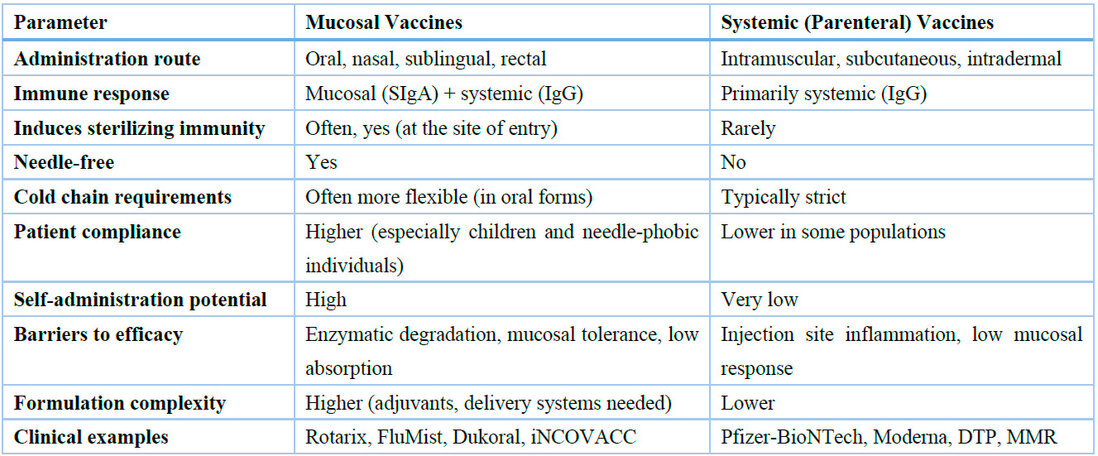
Figure 2. Summary of key adjuvant and delivery strategies for mucosal vaccines. These include detoxified bacterial toxins (e.g., cholera toxin, CpG), Toll-like receptor agonists (e.g., TLR4 activators, flagellin), mucoadhesive polymers (e.g., chitosan, alginate), nanoparticle-based systems (e.g., liposomes, PLGA), bacteriophage vectors (e.g., T4, MS2), and mucosal cytokine adjuvants (e.g., IL-1, IL-17). These technologies enhance antigen uptake, epithelial penetration, and immune activation at mucosal surfaces.
1. Bacterial Toxin Derivatives
Among the most well-studied mucosal adjuvants are detoxified bacterial toxins such as heat-labile (LT) from Escherichia coli and cholera toxin (CT) from Vibrio cholerae 23. Modified versions like dmLT (double mutant LT) and mmCT (multiple mutant CT) retain strong immunostimulatory properties while reducing toxicity 24. These adjuvants enhance antigen uptake by opening tight junctions, promoting dendritic cell activation, and inducing SIgA responses 25. For example, dmLT combined with oral or sublingual antigens has enhanced Th17 and SIgA responses in animal models 26.
2. Toll-like Receptor (TLR) Agonists
TLR agonists represent a promising class of mucosal adjuvants due to their ability to mimic pathogen-associated molecular patterns (PAMPs) and activate innate immunity. Monophosphoryl lipid A (MPL, a TLR4 agonist) is already used in licensed systemic vaccines (e.g., Cervarix®) and is under evaluation for mucosal applications 27. When administered intranasally, TLR9 agonists like CpG oligodeoxynucleotides have enhanced mucosal IgA and systemic IgG responses 28.
3. Mucoadhesive Polymers
Mucoadhesive agents such as chitosan and carboxymethylcellulose prolong antigen contact with mucosal surfaces, improving uptake and stability. Chitosan interacts with mucins to form bioadhesive gels, enhances epithelial permeability, and has shown adjuvanticity in intranasal influenza vaccines 29,30. These polymers also allow nanoparticle co-formulation, facilitating antigen protection and sustained release.
4. Nanoparticle-Based Delivery Systems
Nanocarriers—such as liposomes, PLGA nanoparticles, and virus-like particles (VLPs)—enable targeted delivery and immune cell uptake 31. Particle size and surface charge are critical; particles between 100–200 nm are preferentially taken up by M and dendritic cells, stimulating robust mucosal and systemic responses 32. Lipid-based vesicles, such as liposomes and ISCOMs, have also shown promise in oral and nasal vaccine delivery 33.
5. Recombinant and Viral Vectors
Recombinant vectors such as adenovirus (e.g., Ad5, ChAd) and bacteriophage-based systems provide effective antigen presentation while protecting cargo from degradation 34. Bacteriophage T4-based platforms have recently been shown to induce sterilizing mucosal immunity against SARS-CoV-2 in preclinical models without compromising safety or stability 35.
6. Cytokine-Based Adjuvants
Certain cytokines—including IL-1β, IL-12, and IL-7—have been investigated as mucosal adjuvants to recruit immune cells and modulate local immune responses 36. For instance, intravaginal administration of recombinant IL-7 in non-human primates enhanced mucosal chemokine expression and immune cell homing, supporting its use as a potent mucosal immunomodulator 37.
These advances in adjuvant design and delivery technology have significantly improved the immunogenicity of mucosal vaccines and offer promising pathways toward clinical translation.
However, further human trials are needed to optimize safety profiles and regulatory approval pathways. The key differences between mucosal and systemic vaccines are systematically
compared in Table 2.
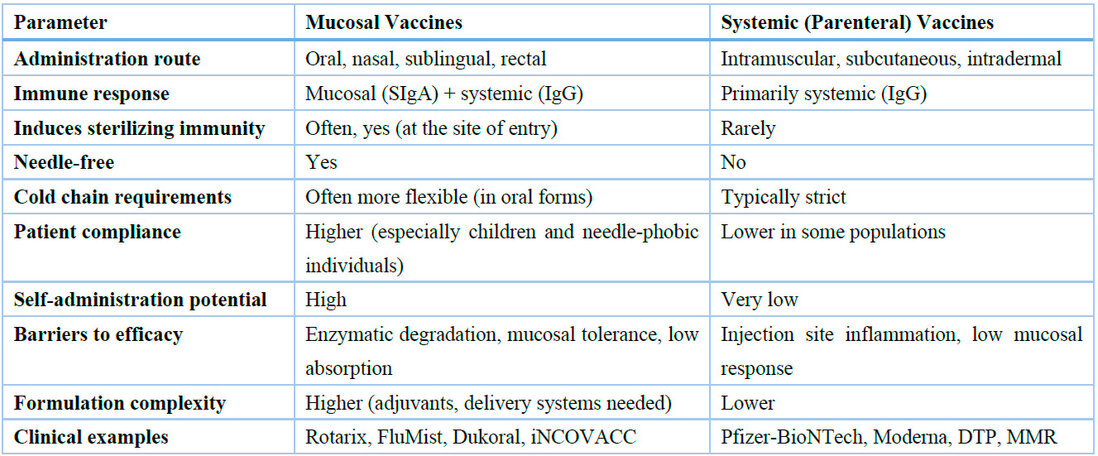
Table 2. Comparative summary of key characteristics of mucosal and systemic vaccines. This table highlights the strengths and limitations of each approach and supports the rationale for expanding mucosal vaccination strategies.
DISCUSSION
Mucosal vaccines hold transformative potential in infectious disease prevention by targeting immune responses at the body's most vulnerable entry points. Unlike traditional systemic immunization, mucosal vaccines can provide localized SIgA-mediated immunity and systemic protection, offering a dual barrier against pathogen colonization and dissemination 4,10,25. This unique immunological profile allows mucosal vaccines to induce sterilizing immunity, which is particularly valuable for preventing transmission during pandemics or in high-risk environments 38. One notable application of mucosal vaccine development is tuberculosis (TB), a respiratory disease ideally suited for mucosal immunization due to its pulmonary transmission route. A promising candidate mucosal vaccine expressing the antigen 85B of Mycobacterium tuberculosis, delivered intranasally in a murine model, demonstrated strong induction of secretory IgA, elevated Th1 cytokine levels in bronchoalveolar lavage fluid, and significant reductions in bacterial burden in both lungs and spleen. These findings illustrate the potential of intranasal vaccination strategies to prevent initial infection and limit systemic dissemination of M. tuberculosis, aligning with the central goal of mucosal vaccines: to block pathogens at their point of entry and achieve sterilizing immunity in diseases spread by aerosol transmission 38.
In addition to immunological benefits, mucosal vaccines present compelling logistical advantages, including needle-free administration, improved patient compliance, and suitability for self-administration and mass campaigns in resource-limited settings 5,7,9. However, antigen stability, mucosal permeability, and consistent immunogenicity challenges persist across diverse populations 13,22. Biological barriers such as acidic environments, mucus viscosity, and mucociliary clearance hinder antigen access to inductive immune sites 31.
Emerging technologies—including mucoadhesive polymers, bacterial toxin derivatives, cytokine-based adjuvants, and nanocarrier-based delivery systems—are actively being developed to address these barriers 23,24,27,32. Some platforms, such as bacteriophage-based vaccines or virus-like particles, have shown preclinical success in achieving mucosal and systemic protection with high safety margins 34,35.
Mucosal adjuvants function by overcoming physicochemical (e.g., low pH, enzymatic degradation) and immunological barriers (e.g., mucosal tolerance) using targeted molecular mechanisms.
For example, the B subunits of bacterial toxins such as LT and CT bind to GM1 gangliosides on epithelial cells, facilitating antigen uptake via endocytosis and stimulating dendritic cell activation through cAMP/PKA signaling pathways 12,13. Engineered mutants such as dmLT and mmCT reduce enterotoxicity while retaining the ability to promote Th17-polarizing cytokines like IL-17 and IL-22—key drivers of mucosal IgA production 15,16,18.
Similarly, Toll-like receptor (TLR) agonists such as CpG (TLR9) and MPL (TLR4) activate antigen-presenting cells (APCs) through the MyD88/NF-κB signaling cascade 19,20. This leads to the secretion of pro-inflammatory cytokines, including IL-6 and TNF-α, promoting APC maturation and the induction of IgA responses in mucosa-associated lymphoid tissues (e.g., GALT, BALT) 21,22,25.
Nanoparticle-based delivery systems—particularly those made of PLGA or liposomes—further enhance mucosal immunogenicity by protecting the antigen cargo, controlling its release over time, and promoting uptake by M cells and APCs due to their optimized size (100–200 nm) and surface charge 34. Positively charged particles exhibit enhanced mucoadhesion and translocation across the mucosal epithelium 31,42.
Innovative hybrid systems combining polymeric nanoparticles with molecular adjuvants (e.g., CpG or dmLT) are being developed to synergize multiple immune pathways 24,25,35. These strategies aim to achieve durable and site-specific mucosal immunity while accounting for interindividual variability due to microbiota composition or enzymatic degradation (Figure 3) 44.
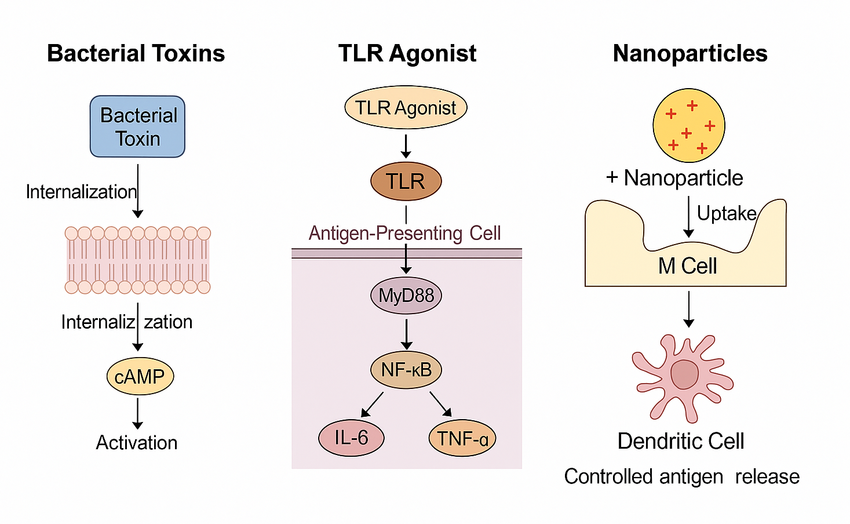
Figure 3. Molecular mechanisms of mucosal adjuvants. Bacterial toxin derivatives bind to GM1 ganglioside receptors, triggering cAMP-dependent pathways that facilitate epithelial permeability and immune activation. TLR agonists stimulate antigen-presenting cells through the MyD88/NF-κB pathway, inducing IL-6 and TNF-α production. Nanoparticles (~100–200 nm, positively charged) are taken up by M and dendritic cells, enabling controlled antigen release and enhanced mucosal immune responses.
Despite these advances, regulatory pathways for mucosal vaccines remain underdeveloped. Most current licensures are limited to oral and intranasal routes, with few sublingual or rectal vaccines reaching clinical trials 16,39. Furthermore, the lack of standardized correlates of protection for mucosal immunity complicates efficacy assessment in humans 42. Investment in translational studies, particularly Phase I/II trials focusing on immune correlates and delivery optimization, will be crucial for future clinical implementation.
Future Directions
The field of mucosal vaccinology is poised for rapid expansion, yet several knowledge and implementation gaps must be addressed. First, developing thermostable formulations that can withstand ambient conditions remains critical for deployment in low-resource settings 41. Second, identifying correlates of protection specific to mucosal immunity is urgently needed to support regulatory evaluation and licensure 40. Third, emerging innovative platforms that combine antigen delivery with immune modulation—such as AI-guided epitope mapping and mucosal microbiome-targeted adjuvants—warrant further exploration 42.
Furthermore, integrating mucosal vaccines into pandemic preparedness frameworks, particularly through stockpiling and rapid-deployment strategies, will enhance global response capacity 43.
Finally, increased investment in translational research, including human challenge models and standardized delivery systems, will be essential for bringing next-generation mucosal
vaccines from bench to bedside. Table 3 summarizes leading mucosal adjuvant technologies' mechanisms, advantages, and development status.

Table 3. Comparative characteristics of mucosal adjuvants under development or clinical testing. The table summarizes mechanisms, immune targets, strengths, limitations, and current translational stages, aiding in selecting optimal adjuvant systems for mucosal vaccine formulation.
CONCLUSIONS
Mucosal vaccines represent a highly promising yet underutilized class of immunization strategies. Their potential to generate broad immune coverage, reduce transmission, and improve global vaccine accessibility positions them as central to next-generation vaccinology.
As advances in immunology, formulation science, and delivery platforms converge, mucosal vaccination is expected to transition from a niche innovation to a mainstream approach. Continued interdisciplinary collaboration among immunologists, formulation scientists, and public health experts is essential to unlock their full potential and bring these innovations globally.
Author Contributions: Conceptualization, L.P.P., and F.C.; Methodology, L.P.P.; Investigation, L.P.P., N.A.M.A., and M.X.K.; Resources, F.C.; Writing—original draft preparation, L.P.P.; Writing—review and editing, N.A.M.A., M.X.K., and F.C.; Visualization, L.P.P.; Supervision, F.C.; Project administration, F.C.; Funding acquisition, F.C. All authors have read and agreed to the published version of the manuscript.
Funding: This research was funded by the Agencia Nacional de Investigación y Desarrollo (ANID) – FONDEF, Government of Chile, under project number ID22I10141. The same grant funded the APC.
Conflicts of Interest: The authors declare no conflict of interest.
REFERENCES
- Lavelle EC, Ward RW. Mucosal vaccines—fortifying the frontiers. Nat Rev Immunol. 2022;22(4):236–250. doi:10.1038/s41577-021-00624-5.
- Gao Q, Bao L, Mao H, Wang L, Xu K, Yang M, et al. Intranasal SARS-CoV-2 vaccine with long-term protection. Nature. 2022;602:639–647. doi:10.1038/s41586-022-04596-2.
- Zhu J, Ananthaswamy N, Jain S, Batra H, Tang W-C, Lewry DA, et al. Bacteriophage T4-based mucosal COVID-19 vaccine. Proc Natl Acad Sci U S A. 2022;119(45):e2212950119. doi:10.1073/pnas.2212950119.
- Maruggi G, Zhang C, Li J, Ulmer JB, Yu D. mRNA vaccines for infectious diseases: principles, delivery and clinical translation. Mol Ther. 2019;27(4):757–772. doi:10.1016/j.ymthe.2019.01.020.
- Gong X, Yang L, Li Y, Yao C, Luo Y, Li D, et al. Chitosan-based mucosal adjuvants: sunrise on the ocean. Vaccines. 2022;10(11):1906. doi:10.3390/vaccines10111906.
- Lycke N, Raghavan S. Advances in mucosal vaccines. Curr Opin Immunol. 2023;80:102291. doi:10.1016/j.coi.2023.102291.
- Holmgren J, Czerkinsky C, Lycke N. Challenges in mucosal vaccine development. Vaccine. 2021;39(32):4269–4275. doi:10.1016/j.vaccine.2021.05.071.
- Kiyono H, Yuki Y. Mucosal vaccines: past, present and future. Int Immunol. 2021;33(12):767–774. doi:10.1093/intimm/dxab056.
- Mao C, Liu A, Cao B, Wang L. Virus-like particles for mucosal vaccines. J Nanobiotechnol. 2020;18(1):135. doi:10.1186/s12951-020-00693-z.
- World Health Organization. Global Vaccine Action Plan 2021–2030. Geneva: WHO; 2021.
- Plotkin SA, Rotz LD, Salmon DA, Hotez PJ. WHO position on rabies vaccines. Vaccine. 2023;41(12):1970–1972. doi:10.1016/j.vaccine.2023.02.011.
- Burnett E, Parashar UD, Tate JE. Rotavirus vaccination impact in low and middle-income countries. Clin Infect Dis. 2020;71(2):316–323. doi:10.1093/cid/ciz868.
- Freytag LC, Clements JD. Mucosal adjuvants: 2023 update. Vaccine. 2023;41(5):1011–1022. doi:10.1016/j.vaccine.2022.12.038.
- Logerot S, Chaumeil C, Brezar V, Roguet G, Demotz S. IL-7 and mucosal immunity. Front Immunol. 2021;12:614115. doi:10.3389/fimmu.2021.614115.
- Miquel-Clopés A, Bentley EG, Stewart JP, Carding SR. Mucosal vaccine technologies. Clin Exp Immunol. 2019;196(2):205–214. doi:10.1111/cei.13285.
- Skwarczynski M, Toth I. Peptide-based synthetic vaccines for the induction of mucosal immunity. Ther Deliv. 2017;8(3):141–156. doi:10.4155/tde-2016-0065.
- Azegami T, Yuki Y, Kiyono H. Challenges in mucosal vaccines for infectious diseases. Int Immunol. 2014;26(9):517–528. doi:10.1093/intimm/dxu063.
- Neutra MR, Kozlowski PA. Mucosal vaccines: the promise and the challenge. Nat Rev Immunol. 2006;6(2):148–158. doi:10.1038/nri1777.
- Czerkinsky C, Holmgren J. Mucosal delivery routes for optimal immunization: targeting immunity to the right tissues. Curr Top Microbiol Immunol. 2012;354:1–18. doi:10.1007/82_2011_155.
- Patel M, Pedreira C, De Oliveira LH, Tate JE, Orozco M, Mercado J, et al. Real-world impact of rotavirus vaccination. Lancet Infect Dis. 2012;12(7):561–570. doi:10.1016/S1473-3099(12)70029-2.
- Ambrose CS, Wu X, Jones T, Mallory RM. The efficacy of intranasal live attenuated influenza vaccine in children. Pediatr Infect Dis J. 2012;31(1):5–9. doi:10.1097/INF.0b013e3182367662.
- Maki J, Guiot AL, Aubert M, Brochier B, Cliquet F, Hanlon CA, et al. Oral vaccination of wildlife using a vaccinia–rabies-glycoprotein recombinant virus vaccine (RABORAL V-RG®): a global review. Vet Res. 2017;48(1):57. doi:10.1186/s13567-017-0459-9.
- Davis R, Jayappa H, Abdelmagid OY, Glaser AL. Comparison of the mucosal immune response in dogs vaccinated with either an intranasal avirulent live culture or a subcutaneous antigen extract vaccine of Bordetella bronchiseptica. Vet Ther. 2007;8(1):32–40. PMID:17472769.
- Day MJ, Horzinek MC, Schultz RD, Squires RA. Pet vaccination strategies: current practice and future considerations. J Small Anim Pract. 2016;57(9):439–442. doi:10.1111/jsap.12467.
- Ottsjö LS, Karlsson KH, Telemo E, Lebens M, Schön E, Eriksson K. dmLT adjuvant improves immunity against H. pylori. Infect Immun. 2013;81(5):1532–1540. doi:10.1128/IAI.01407-12.
- Garçon N, Van Mechelen M. Recent clinical experience with vaccines using MPL- and QS-21-containing adjuvant systems. Expert Rev Vaccines. 2011;10(4):471–486. doi:10.1586/erv.11.29.
- Tighe H, Takabayashi K, Schwartz D, Van Nest G, Tuck S, Eiden JJ, et al. Conjugation of protein to CpG DNA enhances antigen-specific mucosal immunity. J Immunol. 2000;164(5):2635–2643. doi:10.4049/jimmunol.164.5.2635.
- Szymańska E, Winnicka K. Stability of chitosan—a challenge for pharmaceutical and biomedical applications. Mar Drugs. 2015;13(4):1819–1846. doi:10.3390/md13041819.
- Pawar D, Mangal S, Goswami R, Jaganathan KS. Development and characterization of mucoadhesive nanoparticles for nasal delivery of ketorolac tromethamine. Int J Nanomedicine. 2010;5:807–815. doi:10.2147/IJN.S12272.
- Kayamuro H, Yoshioka Y, Abe Y, Arita S, Katayama K, Nomura T, et al. Interleukin-1 as an effective adjuvant for influenza vaccine. J Virol. 2010;84(24):12703–12712. doi:10.1128/JVI.01182-10.
- Chen D, Kristensen D. Opportunities and challenges of developing thermostable vaccines. Expert Rev Vaccines. 2009;8(5):547–557. doi:10.1586/erv.09.21.
- Mestecky J, Moldoveanu Z, Russell MW. Immunologic correlates of protection for mucosal vaccines. Vaccine. 2016;34(41):5205–5212. doi:10.1016/j.vaccine.2016.08.079.
- Levine MM. Needle-free vaccines: an overview and update. Lancet Infect Dis. 2011;11(10):722–731. doi:10.1016/S1473-3099(11)70194-7.
- Gavi, WHO. Vaccine Investment Strategy 2021–2025. Geneva: Gavi & WHO; 2021.
- Vela Ramirez JE, Sharpe LA, Peppas NA. Current state and future trends in nanoparticle-based mucosal vaccine delivery. ACS Nano. 2023;17(8):7067–7091. doi:10.1021/acsnano.3c01234.
- Zhang NN, Zhang L, Deng YQ, Liu Y, Wang Y, Yang M, et al. Next-generation mucosal COVID-19 vaccines. Cell. 2022;185(19):3723–3738. doi:10.1016/j.cell.2022.08.026.
- Walls AC, Miranda MC, Schafer A, Greaney AJ, Guttman M, Zepeda SK, et al. Eliciting immunity at mucosal surfaces. Annu Rev Immunol. 2024;42:1–25. doi:10.1146/annurev-immunol-080223-044323.
- Mohd Asri NA, Xuan KM, Suppian R, Mohd Nor N, Azlan M, Camacho F. Tuberculosis (TB) mucosal vaccines: current efforts and future approaches. Asian J Med Biomed Sci. 2022;6(S1):190–191. Available from: https://journal.unisza.edu.my/ajmb/index.php/ajmb/article/view/582.
- Wang JW, Roden RBS, Wu TC. Plant-derived virus-like particles for mucosal vaccines. Trends Biotechnol. 2022;40(7):798–812. doi:10.1016/j.tibtech.2021.12.005.
- Pardi N, Hogan MJ, Weissman D. Recent advances in mRNA vaccines for infectious diseases. Nat Rev Drug Discov. 2023;22(6):449–475. doi:10.1038/s41573-023-00720-7.
- van Doremalen N, Purushotham JN, Schulz JE, Holbrook MG, Bushmaker T, Carmody A, et al. Intranasal ChAdOx1 nCoV-19 vaccine in rhesus macaques. Sci Transl Med. 2024;16(730):eadj3312. doi:10.1126/scitranslmed.adj3312.
- Mohn KG, Brokstad KA, Cox RJ. Mucosal IgA responses in vaccine development. Front Immunol. 2023;14:1238456. doi:10.3389/fimmu.2023.1238456.
- Tregoning JS, Kinnear E. Respiratory mucosal immunity and vaccine development. Immunity. 2023;56(6):1164–1186. doi:10.1016/j.immuni.2023.05.011.
- Knisely JM, Trautz A, Kumar S, Arruda LB, Smith DM. Synthetic adjuvants for mucosal vaccines. Nat Biotechnol. 2024;42(2):224–237. doi:10.1038/s41587-023-02043-9.
- Garcia-Bennett AE, Alvarado V, Ramírez-García G, Hosta-Rigau L. Nanomaterials for vaccine delivery. Chem Soc Rev. 2024;53(1):338–370. doi:10.1039/D3CS00518E.
Received: March 1, 2025 / Accepted: April 20, 2025 / Published: June 15, 2025
Citation: Padilla Pérez L, Mohd Asri NA, Keh MX, Camacho F. Seal the entry, win the war: mucosal vaccines for a safer tomorrow. Bionatura Journal. 2025;2(2):12. doi:10.70099/BJ/2025.02.02.12
Peer Review Information:
Bionatura Journal thanks the anonymous reviewers for their valuable contribution to the peer review process of this work, supported via https://reviewerlocator.webofscience.com/
Bionatura Journal thanks the anonymous reviewers for their valuable contribution to the peer review process of this work, supported via https://reviewerlocator.webofscience.com/
ISSN: 3020-7886
Publisher's Note:
Bionatura Journal remains neutral with regard to jurisdictional claims in published maps and institutional affiliations.
Bionatura Journal remains neutral with regard to jurisdictional claims in published maps and institutional affiliations.
Copyright:
© 2025 by the authors. This article is published under the terms of the Creative Commons Attribution License (CC BY 4.0), which permits unrestricted use, distribution, and reproduction in any medium, provided the original work is properly cited. For more information, visit: https://creativecommons.org/licenses/by/4.0/
© 2025 by the authors. This article is published under the terms of the Creative Commons Attribution License (CC BY 4.0), which permits unrestricted use, distribution, and reproduction in any medium, provided the original work is properly cited. For more information, visit: https://creativecommons.org/licenses/by/4.0/